355
Activation of cannabinoid CB1 receptors modulates evoked action potentials in rat retinal ganglion cells
JIANG Shu-Xia, LI Qian, WANG Xiao-Han, LI Fang, WANG Zhong-Feng*
Institutes of Brain Science, Institute of Neurobiology and State Key Laboratory of Medical Neurobiology, Fudan University, Shanghai 200032, China
Abstract: Activation of cannabinoid CB1 receptors (CB1Rs) regulates a variety of physiological functions in the vertebrate retina through modulating various types of ion channels. The aim of the present study was to investigate the effects of this receptor on cell excitability of rat retinal ganglion cells (RGCs) in retinal slices using whole-cell patch-clamp techniques. The results showed that under current-clamped condition perfusing WIN55212-2 (WIN, 5 μmol/L), a CB1R agonist, did not significantly change the spontane- ous firing frequency and resting membrane potential of RGCs. In the presence of cocktail synaptic blockers, including excitatory post- synaptic receptor blockers CNQX and D-APV, and inhibitory receptor blockers bicuculline and strychnine, perfusion of WIN (5 μmol/L) hardly changed the frequencies of evoked action potentials by a series of positive current injection (from +10 to +100 pA). Phase- plane plot analysis showed that both average threshold voltage for triggering action potential and delay time to reach threshold voltage were not affected by WIN. However, WIN significantly decreased +dV/dtmax and −dV/dtmax of action potentials, suggestive of reduced rising and descending velocities of action potentials. The effects of WIN were reversed by co-application of SR141716, a CB1R selective antagonist. Moreover, WIN did not influence resting membrane potential of RGCs with synaptic inputs being blocked. These results suggest that activation of CB1Rs may regulate intrinsic excitability of rat RGCs through modulating evoked action potentials.
Key words: action potential; cannabinoid CB1 receptor; patch-clamp; retinal ganglion cell; spontaneous firing; WIN55212-2
大麻素CB1受体对大鼠视网膜神经节细胞诱发动作电位的作用 蒋淑霞,李 倩,王霄汉,李 芳,王中峰*
复旦大学脑科学研究院,神经生物学研究所,医学神经生物学国家重点实验室,上海 200032
摘 要:激活大麻素CB1受体(CB1Rs)通过调控多种离子通道,从而调节脊椎动物视网膜的功能。本文旨在利用膜片钳全细 胞记录技术,在大鼠视网膜薄片上研究CB1Rs对神经节细胞兴奋性的作用。结果显示,在电流钳制状态下,灌流CB1R激动 剂WIN55212-2 (WIN, 5 μmol/L)对神经节细胞的自发动作电位发放频率和静息膜电位均没有显著影响。在灌流液中加入 CNQX,D-APV,bicuculline和strychnine以阻断神经节细胞的兴奋性和抑制性输入,灌流5 μmol/L WIN对正向电流注入(+10 pA到+100 pA)诱发的动作电位的频率也没有显著影响。位相分析结果显示,触发动作电位的阈值电位和触发第一个动作电 位的延迟时间在加入WIN前后也没有显著改变;然而,WIN显著降低动作电位的上升和下降相速率(±dV/dtmax),而且该作 用可被CB1R拮抗剂SR141716所阻断。此外,在阻断突触输入的情况下,WIN对神经节细胞的膜电位也没有显著影响。以上 结果提示,激活大麻素CB1Rs通过调控诱发动作电位,从而调节大鼠视网膜神经节细胞的兴奋性。
关键词:动作电位;大麻素CB1受体;膜片钳;神经节细胞;自发动作电位发放;WIN55212-2 中图分类号:Q424;Q436
Received 2013-03-25 Accepted 2013-05-17
This work was supported by the National Basic Research Development Program of China (No. 2013CB835101), the National Natural Science Foundation of China (No. 30930034), the Key Research Program of Science and Technology Commissions of Shanghai Munici- pality, China (No. 11JC1401200) and Research Fund for the Doctoral Program of Higher Education of China (No. 20110071110031).
JIANG Shu-Xia, LI Qian, WANG Xiao-Han, LI Fang, WANG Zhong-Feng*
Institutes of Brain Science, Institute of Neurobiology and State Key Laboratory of Medical Neurobiology, Fudan University, Shanghai 200032, China
Abstract: Activation of cannabinoid CB1 receptors (CB1Rs) regulates a variety of physiological functions in the vertebrate retina through modulating various types of ion channels. The aim of the present study was to investigate the effects of this receptor on cell excitability of rat retinal ganglion cells (RGCs) in retinal slices using whole-cell patch-clamp techniques. The results showed that under current-clamped condition perfusing WIN55212-2 (WIN, 5 μmol/L), a CB1R agonist, did not significantly change the spontane- ous firing frequency and resting membrane potential of RGCs. In the presence of cocktail synaptic blockers, including excitatory post- synaptic receptor blockers CNQX and D-APV, and inhibitory receptor blockers bicuculline and strychnine, perfusion of WIN (5 μmol/L) hardly changed the frequencies of evoked action potentials by a series of positive current injection (from +10 to +100 pA). Phase- plane plot analysis showed that both average threshold voltage for triggering action potential and delay time to reach threshold voltage were not affected by WIN. However, WIN significantly decreased +dV/dtmax and −dV/dtmax of action potentials, suggestive of reduced rising and descending velocities of action potentials. The effects of WIN were reversed by co-application of SR141716, a CB1R selective antagonist. Moreover, WIN did not influence resting membrane potential of RGCs with synaptic inputs being blocked. These results suggest that activation of CB1Rs may regulate intrinsic excitability of rat RGCs through modulating evoked action potentials.
Key words: action potential; cannabinoid CB1 receptor; patch-clamp; retinal ganglion cell; spontaneous firing; WIN55212-2
大麻素CB1受体对大鼠视网膜神经节细胞诱发动作电位的作用 蒋淑霞,李 倩,王霄汉,李 芳,王中峰*
复旦大学脑科学研究院,神经生物学研究所,医学神经生物学国家重点实验室,上海 200032
摘 要:激活大麻素CB1受体(CB1Rs)通过调控多种离子通道,从而调节脊椎动物视网膜的功能。本文旨在利用膜片钳全细 胞记录技术,在大鼠视网膜薄片上研究CB1Rs对神经节细胞兴奋性的作用。结果显示,在电流钳制状态下,灌流CB1R激动 剂WIN55212-2 (WIN, 5 μmol/L)对神经节细胞的自发动作电位发放频率和静息膜电位均没有显著影响。在灌流液中加入 CNQX,D-APV,bicuculline和strychnine以阻断神经节细胞的兴奋性和抑制性输入,灌流5 μmol/L WIN对正向电流注入(+10 pA到+100 pA)诱发的动作电位的频率也没有显著影响。位相分析结果显示,触发动作电位的阈值电位和触发第一个动作电 位的延迟时间在加入WIN前后也没有显著改变;然而,WIN显著降低动作电位的上升和下降相速率(±dV/dtmax),而且该作 用可被CB1R拮抗剂SR141716所阻断。此外,在阻断突触输入的情况下,WIN对神经节细胞的膜电位也没有显著影响。以上 结果提示,激活大麻素CB1Rs通过调控诱发动作电位,从而调节大鼠视网膜神经节细胞的兴奋性。
关键词:动作电位;大麻素CB1受体;膜片钳;神经节细胞;自发动作电位发放;WIN55212-2 中图分类号:Q424;Q436
Received 2013-03-25 Accepted 2013-05-17
This work was supported by the National Basic Research Development Program of China (No. 2013CB835101), the National Natural Science Foundation of China (No. 30930034), the Key Research Program of Science and Technology Commissions of Shanghai Munici- pality, China (No. 11JC1401200) and Research Fund for the Doctoral Program of Higher Education of China (No. 20110071110031).
*Corresponding author. Tel: +86-21-54237810; Fax: +86-21-54237643; E-mail: zfwang@fudan.edu.cn
pg. 356
Cannabinoid CB1 receptor (CB1R), a G-protein cou- pled receptor, which is extensively distributed through- out the central nervous system (CNS) [1–3], plays impor- tant roles in regulating multiple neuronal functions, including learning and memory, synaptic plasticity, pain response, and many intracellular signaling path- ways [4–8]. Growing evidence has demonstrated that CB1R signaling is also widely expressed in the verte- brate retina [9, 10]. CB1Rs and endocannabinoids (eCBs), including anandamide (AEA) and 2-Arachidonoylglyc- erol (2-AG), as well as an eCB degradative enzyme, fatty acid amide hydrolase (FAAH), were found in a variety of retinal cell populations and in the inner plex- iform layer (IPL) [11–15], suggesting that eCBs may regu- late the functions of retinal neurons and participate in multiple circuits of visual information processing. For example, WIN55212-2 (WIN), a CB1R agonist, modu- lated various types of voltage-gated K+ and Ca2+ currents in retinal photoreceptors and bipolar cells (BCs) [13, 15–17]. Retrograde modulation of glutamate release from cones by 2-AG was found in goldfish [18].
Retinal ganglion cells (RGCs), output neurons of the retina, receive inhibitory inputs from GABAergic and glycinergic amacrine cells (ACs) mediated by GABAA and glycine receptors and excitatory inputs from BCs mediated by AMPA receptors [19–22]. CB1Rs have been found in BC, ACs and RGCs [11–15]. Activation of CB1Rs in these cells may modulate RGCs excitability, thus influencing visual information processing. How- ever, the effects of CB1R signaling on RGCs are poorly understood. The only evidence was from the cultured RGCs that WIN inhibited Ca2+ currents in these cells [11]. In the present work, we studied the effects of WIN on RGC excitability using patch-clamp techniques in rat retinal slices.
Retinal ganglion cells (RGCs), output neurons of the retina, receive inhibitory inputs from GABAergic and glycinergic amacrine cells (ACs) mediated by GABAA and glycine receptors and excitatory inputs from BCs mediated by AMPA receptors [19–22]. CB1Rs have been found in BC, ACs and RGCs [11–15]. Activation of CB1Rs in these cells may modulate RGCs excitability, thus influencing visual information processing. How- ever, the effects of CB1R signaling on RGCs are poorly understood. The only evidence was from the cultured RGCs that WIN inhibited Ca2+ currents in these cells [11]. In the present work, we studied the effects of WIN on RGC excitability using patch-clamp techniques in rat retinal slices.
1 MAteRiAlsAndMethods
All experimental procedures with animals described in the present work were in accordance with the National Institutes of Health (NIH) guidelines for the Care and Use of Laboratory Animals and the guidelines of Fudan University on the ethical use of animals. During this study all efforts were made to minimize the number of animals used and their suffering. Male Sprague-Dawley rats, aged 21–28 d and obtained from SLAC Laboratory Animal Co., Ltd (Shanghai, China), were housed under conditions of a 12 h/12 h light/dark cycle.
All experimental procedures with animals described in the present work were in accordance with the National Institutes of Health (NIH) guidelines for the Care and Use of Laboratory Animals and the guidelines of Fudan University on the ethical use of animals. During this study all efforts were made to minimize the number of animals used and their suffering. Male Sprague-Dawley rats, aged 21–28 d and obtained from SLAC Laboratory Animal Co., Ltd (Shanghai, China), were housed under conditions of a 12 h/12 h light/dark cycle.
1.1 Preparation of retinal slices
The rats were deeply anesthetized with urethane (25 mg/mL) and sacrificed by decapitation. Eyes were enu- cleated quickly and immersed in ice-cold artificial cere- brospinal fluid (ACSF) containing (in mmol/L): 125 NaCl, 3 KCl, 26 NaHCO3, 1.25 NaH2PO4, 2 CaCl2, 1 MgCl2, 15 glucose (pH 7.4), bubbled with 95% O2 and 5% CO2. Retinae were then isolated and sliced ver- tically in a thickness of 200 μm on a Narishige slicer (ST-20-P, Tokyo, Japan). Slices were transferred to a holding chamber where they completely submerged in oxygenated ACSF solution and maintained at 33–34 oC for 30 min before recording.
1.2 Electrophysiological recordings
Whole-cell current-clamp recordings were performed using standard techniques [23, 24]. Individual slices were transferred to a perfusing chamber and continuously superfused with oxygenated ACSF at a rate of 1–2 mL/ min at 33–34 oC. RGCs in retinal slices were identified by their locations and morphology with the help of an infrared-differential interference contrast (IR-DIC) vid- eo microscopy (Olympus, Japan), and further identified by intracellular injection of Lucifer Yellow. Patch pi- pettes were made by pulling BF150-86-10 glass (Sutter Instrument Co., Novato, CA, USA) on a P-97 Flaming/ Brown micropipette puller (Sutter Instrument) and fire polished (Model MF-830, Narishige, Japan) before re- cording. The pipette resistance was typically 4–8 MΩ after filled with the internal solution (in mmol/L): 120 potassium D-gluconate, 1 ethylene glycol-bis(β- aminoethyl ether) N, N, N’, N’-tetraacetic acid (EGTA), 10 4-(2-Hydroxyethyl)piperazine-1-ethane- sulfonic acid (HEPES), 4 ATP-Mg, 0.3 GTP-Na, 10 phosphocreatine, 0.1 CaCl2, 1 MgCl2, 5 Lucifer Yellow, pH 7.2 adjusted with KOH and to 280–290 mOsm/L. Whole-cell membrane potential were recorded from RGCs by a patch amplifier (Axopatch 700B; Molecular Devices, Foster City, CA, USA) with Digidata 1440A data acquisition board and pClamp 10.2 software at a sampling rate of 10 kHz and a low-pass filter of 1 kHz.
1.3 Drugs and solutions
Bicuculline, D-(-)-2-amino-5-phosphonopentanoic acid (D-APV) and 6-Cyano-7-nitroquinoxaline-2,3-dione (CNQX) were purchased from Tocris (Tocris Biosci- ence, Ellisville, MO, USA) and SR141716 was from Cayman Chemical (Ann Arbor, MI, USA). All others were from Sigma Chemical Company (Sigma-Aldrich, Inc., St. Louis, MO, USA). CNQX, SR141716 and WIN were first dissolved in dimethyl sulfoxide (DMSO) and then diluted in ACSF solution with a final DMSO concentration less than 0.1%, which has no sig- nificant effects on membrane response of RGCs. All other chemicals were prepared in distilled water, stored at −20 oC and freshly diluted to the final concentration using ACSF solution.
The rats were deeply anesthetized with urethane (25 mg/mL) and sacrificed by decapitation. Eyes were enu- cleated quickly and immersed in ice-cold artificial cere- brospinal fluid (ACSF) containing (in mmol/L): 125 NaCl, 3 KCl, 26 NaHCO3, 1.25 NaH2PO4, 2 CaCl2, 1 MgCl2, 15 glucose (pH 7.4), bubbled with 95% O2 and 5% CO2. Retinae were then isolated and sliced ver- tically in a thickness of 200 μm on a Narishige slicer (ST-20-P, Tokyo, Japan). Slices were transferred to a holding chamber where they completely submerged in oxygenated ACSF solution and maintained at 33–34 oC for 30 min before recording.
1.2 Electrophysiological recordings
Whole-cell current-clamp recordings were performed using standard techniques [23, 24]. Individual slices were transferred to a perfusing chamber and continuously superfused with oxygenated ACSF at a rate of 1–2 mL/ min at 33–34 oC. RGCs in retinal slices were identified by their locations and morphology with the help of an infrared-differential interference contrast (IR-DIC) vid- eo microscopy (Olympus, Japan), and further identified by intracellular injection of Lucifer Yellow. Patch pi- pettes were made by pulling BF150-86-10 glass (Sutter Instrument Co., Novato, CA, USA) on a P-97 Flaming/ Brown micropipette puller (Sutter Instrument) and fire polished (Model MF-830, Narishige, Japan) before re- cording. The pipette resistance was typically 4–8 MΩ after filled with the internal solution (in mmol/L): 120 potassium D-gluconate, 1 ethylene glycol-bis(β- aminoethyl ether) N, N, N’, N’-tetraacetic acid (EGTA), 10 4-(2-Hydroxyethyl)piperazine-1-ethane- sulfonic acid (HEPES), 4 ATP-Mg, 0.3 GTP-Na, 10 phosphocreatine, 0.1 CaCl2, 1 MgCl2, 5 Lucifer Yellow, pH 7.2 adjusted with KOH and to 280–290 mOsm/L. Whole-cell membrane potential were recorded from RGCs by a patch amplifier (Axopatch 700B; Molecular Devices, Foster City, CA, USA) with Digidata 1440A data acquisition board and pClamp 10.2 software at a sampling rate of 10 kHz and a low-pass filter of 1 kHz.
1.3 Drugs and solutions
Bicuculline, D-(-)-2-amino-5-phosphonopentanoic acid (D-APV) and 6-Cyano-7-nitroquinoxaline-2,3-dione (CNQX) were purchased from Tocris (Tocris Biosci- ence, Ellisville, MO, USA) and SR141716 was from Cayman Chemical (Ann Arbor, MI, USA). All others were from Sigma Chemical Company (Sigma-Aldrich, Inc., St. Louis, MO, USA). CNQX, SR141716 and WIN were first dissolved in dimethyl sulfoxide (DMSO) and then diluted in ACSF solution with a final DMSO concentration less than 0.1%, which has no sig- nificant effects on membrane response of RGCs. All other chemicals were prepared in distilled water, stored at −20 oC and freshly diluted to the final concentration using ACSF solution.
1.4 Data analysis
Data analysis was performed off-line using the Clamp- fit 10.2 (Molecular Devices, Foster City, CA, USA) and MATLAB R2011a (MathWorks, USA). For action po- tential analysis, the temporal derivative dV/dt was first calculated by the equation of the form dV/dt(tn) = (Vn+1 − Vn-1) / 2∆t, and then plotted it against the instanta- neous membrane potential to yield the phase-plane plot [25, 26]. The threshold voltage for triggering the first action potential was determined using a criterion that the velocity of action potential reaching 20 mV/ms. Data are presented as mean ± SEM. Paired t test was used for statistical analysis, and a P value < 0.05 was considered statistically significant.
Data analysis was performed off-line using the Clamp- fit 10.2 (Molecular Devices, Foster City, CA, USA) and MATLAB R2011a (MathWorks, USA). For action po- tential analysis, the temporal derivative dV/dt was first calculated by the equation of the form dV/dt(tn) = (Vn+1 − Vn-1) / 2∆t, and then plotted it against the instanta- neous membrane potential to yield the phase-plane plot [25, 26]. The threshold voltage for triggering the first action potential was determined using a criterion that the velocity of action potential reaching 20 mV/ms. Data are presented as mean ± SEM. Paired t test was used for statistical analysis, and a P value < 0.05 was considered statistically significant.
2 Results
2.1 Win does not change spontaneous firing and membrane potential of RGCs
RGCs display spontaneous firing under physiological condition [27]. We first examined whether activation of CB1Rs may affect spontaneous firing of rat RGCs. As shown in Fig. 1A, perfusion of WIN (5 μmol/L) for 10 min did not change the spontaneous firing frequency in a RGC (upper trace). The average firing frequency (from 9 to 10 min after WIN application) obtained from 10 cells was (0.88 ± 0.49) Hz, which was not signifi- cantly different from that before WIN application (0.40 Hz ± 0.17 Hz) (P = 0.125, Fig. 1B). At the same time, WIN also hardly changed resting membrane potential of the cell (Fig. 1A, lower enlarged trace), with the av- erage being (−56.48 ± 1.09) mV (n = 10, P = 0.356 vs control value of −56.08 mV± 1.23 mV) (Fig. 1C).
2.2 effects of Win on evoked action potentials of rat RGCs
In order to test whether WIN may directly influence intrinsic excitability of rat RGC, we perfused cocktail synaptic blockers, including bicuculline (10 μmol/L), strychnine (10 μmol/L), CNQX (10 μmol/L) and D- APV (50 μmol/L), to obstruct the synaptic inputs, and then examined the effects of WIN on RGCs. Fig. 2A and 2B show membrane responses of a RGC to a series of 500 ms positive injected currents from +10 pA to +100 pA in a 10 pA increment before and 10 min after perfusing 5 μmol/L WIN. The range of injected cur- rents was enough to induce appropriate firing in RGCs. WIN did not remarkably change firing pattern and fre- quency of the cell. Fig. 2C shows representative re- cordings in a +20 pA current injection. Data analysis revealed that the firing frequency of the cells was increased accompanying with increased positive current injection (Fig. 2D). The average firing frequency was increased from (5.76 ± 1.85) Hz to (67.60 ± 3.33) Hz (n = 17) when the injected current was from +10 pA to +100 pA in control, while it was from (7.29 ± 2.45) Hz to (65.47 ± 3.33) Hz (n = 17) after WIN application. There was no significant difference before and after WIN application at all injected current levels (Fig. 2D).
2.1 Win does not change spontaneous firing and membrane potential of RGCs
RGCs display spontaneous firing under physiological condition [27]. We first examined whether activation of CB1Rs may affect spontaneous firing of rat RGCs. As shown in Fig. 1A, perfusion of WIN (5 μmol/L) for 10 min did not change the spontaneous firing frequency in a RGC (upper trace). The average firing frequency (from 9 to 10 min after WIN application) obtained from 10 cells was (0.88 ± 0.49) Hz, which was not signifi- cantly different from that before WIN application (0.40 Hz ± 0.17 Hz) (P = 0.125, Fig. 1B). At the same time, WIN also hardly changed resting membrane potential of the cell (Fig. 1A, lower enlarged trace), with the av- erage being (−56.48 ± 1.09) mV (n = 10, P = 0.356 vs control value of −56.08 mV± 1.23 mV) (Fig. 1C).
2.2 effects of Win on evoked action potentials of rat RGCs
In order to test whether WIN may directly influence intrinsic excitability of rat RGC, we perfused cocktail synaptic blockers, including bicuculline (10 μmol/L), strychnine (10 μmol/L), CNQX (10 μmol/L) and D- APV (50 μmol/L), to obstruct the synaptic inputs, and then examined the effects of WIN on RGCs. Fig. 2A and 2B show membrane responses of a RGC to a series of 500 ms positive injected currents from +10 pA to +100 pA in a 10 pA increment before and 10 min after perfusing 5 μmol/L WIN. The range of injected cur- rents was enough to induce appropriate firing in RGCs. WIN did not remarkably change firing pattern and fre- quency of the cell. Fig. 2C shows representative re- cordings in a +20 pA current injection. Data analysis revealed that the firing frequency of the cells was increased accompanying with increased positive current injection (Fig. 2D). The average firing frequency was increased from (5.76 ± 1.85) Hz to (67.60 ± 3.33) Hz (n = 17) when the injected current was from +10 pA to +100 pA in control, while it was from (7.29 ± 2.45) Hz to (65.47 ± 3.33) Hz (n = 17) after WIN application. There was no significant difference before and after WIN application at all injected current levels (Fig. 2D).
Fig. 1. WIN55212-2 (WIN) does not affect spontaneous firing and resting membrane potential of RGCs in retinal slices with synaptic inputs being intact. A: Representative trace recorded from a RGC, showing that extracellular application of WIN (5 μmol/L) did not affect spontaneous firing and resting membrane potential of the cell. Lower trace is enlarged one to show change of membrane potential. B, C: Bar charts summarizing the chang- es of spontaneous firing frequency (B) and membrane potential (C) of RGCs before and after application of WIN. Data are pre- sented as mean ± SEM, n = 10.
We analyzed the effects of WIN on individual action potential evoked by current injection. For simple pur- pose, we only chose and analyzed the first action poten- tial in each current injection [25, 26]. Fig. 3A shows repre- sentative phase-plane trajectories of the action potentials before (control) and after WIN application. Fig. 3B is an enlarged figure from the square as shown in Fig. 3A, clearly showing unchanged threshold volt- ages. The average threshold voltage for triggering ac- tion potential was from (−41.04 ± 2.14) mV to (−43.15 ± 2.04) mV as injected currents were increased from +10 pA to +100 pA after WIN application, which were not significantly different from those of control (from−41.49 mV± 2.10 mV to −43.93 mV± 2.14 mV, n = 17) (Fig. 3C). Moreover, the delay time from onset of cur- rent injection to the threshold voltage for triggering the first action potential was also unchanged after WIN ap- plication, with the average being from 169.3 ms ± 17.6 ms to 102.3 ms ± 0.9 ms (n = 17) at +10 pA to +100 pA current injection respectively, comparable to the control levels(178.8ms±11.7msto102.9ms±0.8ms,n= 17)(Fig. 3D).
We analyzed the effects of WIN on individual action potential evoked by current injection. For simple pur- pose, we only chose and analyzed the first action poten- tial in each current injection [25, 26]. Fig. 3A shows repre- sentative phase-plane trajectories of the action potentials before (control) and after WIN application. Fig. 3B is an enlarged figure from the square as shown in Fig. 3A, clearly showing unchanged threshold volt- ages. The average threshold voltage for triggering ac- tion potential was from (−41.04 ± 2.14) mV to (−43.15 ± 2.04) mV as injected currents were increased from +10 pA to +100 pA after WIN application, which were not significantly different from those of control (from−41.49 mV± 2.10 mV to −43.93 mV± 2.14 mV, n = 17) (Fig. 3C). Moreover, the delay time from onset of cur- rent injection to the threshold voltage for triggering the first action potential was also unchanged after WIN ap- plication, with the average being from 169.3 ms ± 17.6 ms to 102.3 ms ± 0.9 ms (n = 17) at +10 pA to +100 pA current injection respectively, comparable to the control levels(178.8ms±11.7msto102.9ms±0.8ms,n= 17)(Fig. 3D).
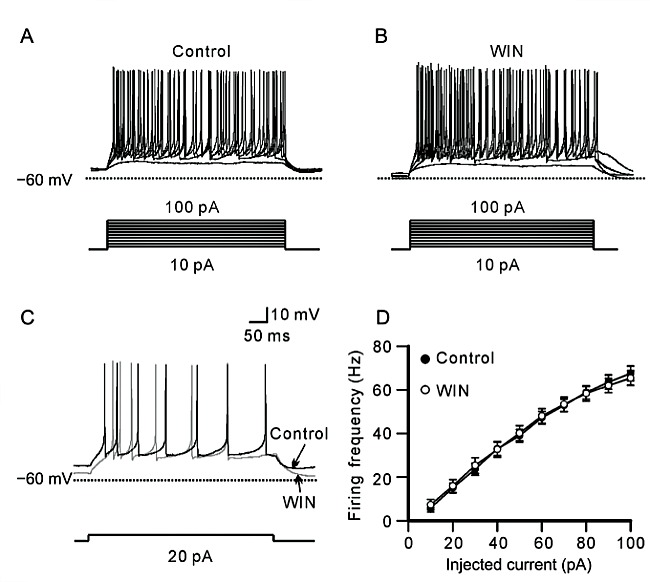
Fig. 2. WIN does not affect firing frequency of evoked action potentials of RGCs with synaptic inputs being blocked. A, B: Represen- tative traces obtained from a RGC, showing the effect of WIN (5 μmol/L) on firing frequency of evoked action potentials before (A) and after application of 5 μmol/L WIN (B). A series of positive currents ranging from +10 pA to +100 pA was injected. C: Sample traces show that 5 μmol/L WIN did not significantly affect firing frequency of action potentials evoked by +20 pA current injection in a RGC. D: Plot of average firing frequency of evoked action potentials versus different injected currents, showing that WIN did not change firing frequency of the action potentials at all injected current levels. Data are presented as mean ± SEM, n = 17.


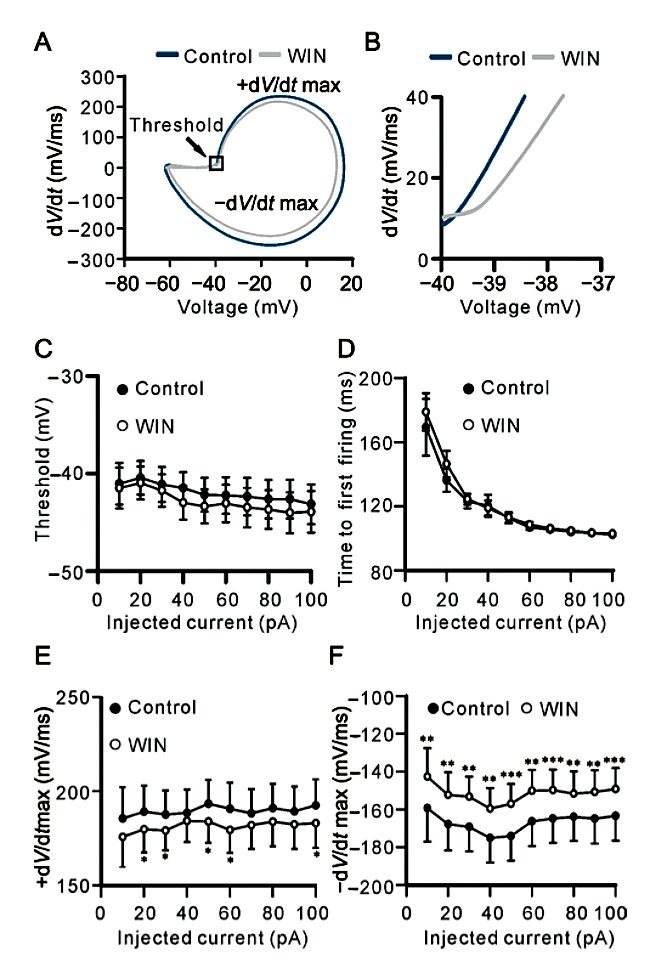
Fig. 3. WIN reduces rising and descending phase velocities of evoked action potential of RGCs. A: Representative phase-plane plots of action potentials before (control) and after 5 μmol/L WIN application. B: Enlarged figure obtained from the square in A, showing that WIN did not change threshold voltage for trig- gering the first action potential of a RGC. C: Plots of average threshold voltage for triggering the first action potential versus different injected currents, showing that WIN did not change the threshold voltage at all injected current levels. D: Plots of aver- age time from onset of current injection to first action potential versus different injected currents, showing that WIN did not change the delay time at all injected current levels. E, F: Plots of average +dV/dtmax and −dV/dtmax of action potentials ver- sus different injected currents, showing that WIN reduced +dV/ dtmax of action potentials at some injected current levels, and −dV/dtmax of action potentials at all injected current levels. Data are presented as mean ± SEM, n = 17. *P < 0.05, **P < 0.01 and ***P < 0.001 vs control.
Although WIN did not influence the threshold volt- age for triggering action potential and the delay time to reach threshold voltage, it is clearly shown from phase- plane plots of the action potentials that WIN may change the rate of both depolarizing and repolarizing phases (Fig. 3A). Pooled data from 17 cells revealed that WIN may somewhat modulate maximum rising phase rate (+dV/dtmax) of the action potentials (Fig. 3E). Specifically, the +dV/dtmax (mV/ms) was signifi- cantly reduced at +20 pA (179.7 ± 12.3 vs 189.1 ± 13.7, P = 0.049), +30 pA(179.1 ± 10.5 vs 187.5 ± 12.8 , P = 0.043), +50 pA (183.8 ± 11.2 vs 193.3 ± 12.7, P =0.046), +60 pA (179.4 ± 12.2 vs 190.7 ± 13.8, P = 0.020) and +100 pA current injection (183.0 ± 13.1 vs 192.4 ± 13.8, P = 0.026), respectively. Meanwhile, WIN remarkably reduced maximum descending phase rate (−dV/dtmax) of the action potentials at all current injected levels (n = 17, P all < 0.01 or 0.001) (Fig. 3F). In addition, WIN-induced effects on ±dV/dtmax of evoked action potentials were eliminated by co-appli- cation of SR141716 (300 nmol/L), a CB1R antagonist. As shown in Table 1 and Table 2, co-applying SR141716 and WIN for 10 min, ±dV/dtmax of action potentials at all current injected levels were comparable to control.
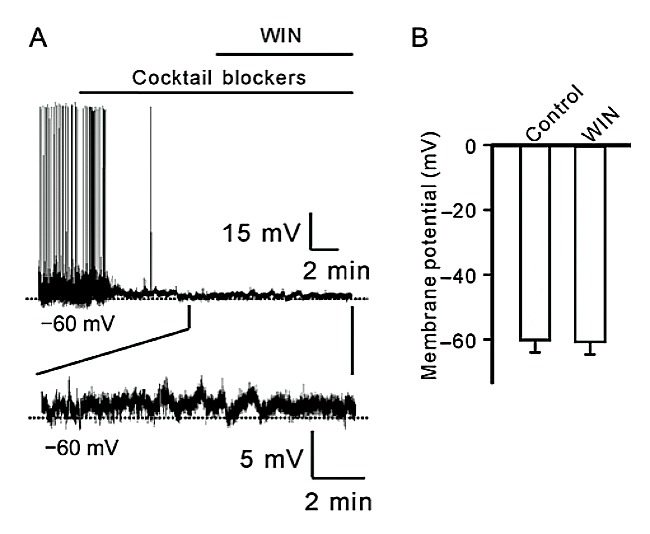
Fig. 4. WIN does not change resting membrane potentials of RGCs with synaptic inputs being blocked. A: Representative trace shows the effect of WIN on resting membrane potential of a RGC. Lower trace is enhanced one to show change of mem- brane potential. Note that WIN did not induce changes of mem- brane potential of the cell. B: Bar chart summarizing the changes of resting membrane potential of RGCs before and after applica- tion of WIN. Data are presented as mean ± SEM, n = 5.
2.3 Win does not change resting membrane poten- tial of RGCs in the absence of synaptic inputs Finally, we tested the effects of WIN on resting mem- brane potential of RGCs in the absence of synaptic inputs. As shown in Fig. 4A, after spontaneous firing was blocked by perfusing cocktail synaptic blockers, WIN (5 μmol/L) was applied to a RGC in retinal slice. WIN failed to induce the cell to firing. Enlarged figure clearly shows that membrane potential of the cell was not significantly changed 10 min after WIN applica- tion. Summary data obtained from 5 cells revealed that the average resting potential was (−61.3 ± 3.9) mV in the presence of WIN, comparable to that of control (−60.8 mV ± 3.9 mV, n = 5, P = 0.138) (Fig. 4B).
3 disCussion
In the inner retina, BCs, ACs and RGCs form a neu- ronal circuit that transmits the visual signals and modu- lates the visual information processing. The excitability of RGCs is determined by the balance of inhibitory and excitatory inputs, as well as the intrinsic properties of the cells. The present results showed that cannabinoid receptor agonist WIN did not significantly change spontaneous firing frequency of rat RGCs with synaptic inputs being intact (Fig. 1), suggesting that WIN may not influence intrinsic electrophysiological properties of RGCs under such condition. This was supported by the fact that WIN had no significant effect on resting membrane potential of RGCs without synaptic inputs being blocked by cocktail synaptic blockers (Fig. 1).
In the inner retina, BCs, ACs and RGCs form a neu- ronal circuit that transmits the visual signals and modu- lates the visual information processing. The excitability of RGCs is determined by the balance of inhibitory and excitatory inputs, as well as the intrinsic properties of the cells. The present results showed that cannabinoid receptor agonist WIN did not significantly change spontaneous firing frequency of rat RGCs with synaptic inputs being intact (Fig. 1), suggesting that WIN may not influence intrinsic electrophysiological properties of RGCs under such condition. This was supported by the fact that WIN had no significant effect on resting membrane potential of RGCs without synaptic inputs being blocked by cocktail synaptic blockers (Fig. 1).
Considering that RGCs receive both inhibitory and ex- citatory inputs from ACs and BCs [19–22], and activation of CB1Rs at the terminals of ACs and BCs by WIN in- hibited spontaneous release of GABA and glutamate [28], WIN may simultaneously modulate inhibitory and excitatory inputs of RGCs, thus not disturbing the bal- ance of inhibitory and excitatory inputs of the cells.
Although WIN did not affect resting membrane po- tential of rat RGCs, it remarkably reduced rising and descending rates of evoked action potentials (Fig. 3). Previous studies have shown that WIN concentration dependently inhibited voltage-gated sodium currents (INa) in cultured rat trigeminal ganglion cells via CB1Rs [29]. AEA also inhibited INa in rat dorsal root ganglion neu- rons, and the effect was not reversed by AM251 and AM630, suggestive of a direct action of AEA on Na+ channels [30]. Na+ channels were expressed in rat RGCs and involved in the generation of rising phase of action potential [29]. WIN-induced reduction of +dV/dtmax of action potential may be due to its inhibitory effect on Na+ channels. Moreover, cannabinoid-induced suppres- sion of Ca2+ channels may also partially contribute to WIN-induced reduction of +dV/dtmax of action poten- tial [11]. Descending phase of action potential is contrib- uted by K+ channels [31, 32]. RGCs expressed multiple types of voltage-gated K+ channels [25, 33–36], and activa- tion of cannabinoid receptors by WIN may modulate various K+ currents in photoreceptors and BCs [13, 15–17]. WIN-induced reduction of −dV/dtmax of action poten- tial may be resulted from its inhibition on K+ channels. Furthermore, WIN-induced effect on action potential was mediated by CB1Rs because SR141716 could re- verse WIN effect. On the other hand, WIN did not change the resting membrane potential and spontane- ous firing of RGCs with or without synaptic inputs be- ing blocked by cocktail synaptic blockers, suggesting that WIN had no modulating roles on the K+ channels, such as inward rectifying (Kir) and small-conductance Ca2+-activated K+ (SKCa) channels, as well as hyperpo- larization-activated cation channels (Ih), which are in- volved in the generation of resting membrane potential and after-hyperpolarization potential.
The present work demonstrated that activation of CB1Rs regulates rat RGC excitability through modulat- ing rising and descending rates of evoked action poten- tials of the cells.
Although WIN did not affect resting membrane po- tential of rat RGCs, it remarkably reduced rising and descending rates of evoked action potentials (Fig. 3). Previous studies have shown that WIN concentration dependently inhibited voltage-gated sodium currents (INa) in cultured rat trigeminal ganglion cells via CB1Rs [29]. AEA also inhibited INa in rat dorsal root ganglion neu- rons, and the effect was not reversed by AM251 and AM630, suggestive of a direct action of AEA on Na+ channels [30]. Na+ channels were expressed in rat RGCs and involved in the generation of rising phase of action potential [29]. WIN-induced reduction of +dV/dtmax of action potential may be due to its inhibitory effect on Na+ channels. Moreover, cannabinoid-induced suppres- sion of Ca2+ channels may also partially contribute to WIN-induced reduction of +dV/dtmax of action poten- tial [11]. Descending phase of action potential is contrib- uted by K+ channels [31, 32]. RGCs expressed multiple types of voltage-gated K+ channels [25, 33–36], and activa- tion of cannabinoid receptors by WIN may modulate various K+ currents in photoreceptors and BCs [13, 15–17]. WIN-induced reduction of −dV/dtmax of action poten- tial may be resulted from its inhibition on K+ channels. Furthermore, WIN-induced effect on action potential was mediated by CB1Rs because SR141716 could re- verse WIN effect. On the other hand, WIN did not change the resting membrane potential and spontane- ous firing of RGCs with or without synaptic inputs be- ing blocked by cocktail synaptic blockers, suggesting that WIN had no modulating roles on the K+ channels, such as inward rectifying (Kir) and small-conductance Ca2+-activated K+ (SKCa) channels, as well as hyperpo- larization-activated cation channels (Ih), which are in- volved in the generation of resting membrane potential and after-hyperpolarization potential.
The present work demonstrated that activation of CB1Rs regulates rat RGC excitability through modulat- ing rising and descending rates of evoked action poten- tials of the cells.
RefeRenCes
1 Hashimotodani Y, Ohno-Shosaku T, Kano M. Endocannabinoids and synaptic function in the CNS. Neuroscientist 2007; 13: 127–137.
2 Herkenham M, Lynn AB, Little MD, Johnson MR, MelvinLS, de Costa BR, Rice KC. Cannabinoid receptor localization in brain. Proc Nat Acad Sci U S A 1990; 87: 1932–1936.
3 Matsuda LA, Lolait SJ, Brownstein MJ, Young AC, Bonner TI. Structure of a cannabinoid receptor and functional expression of the cloned cDNA. Nature 1990; 346: 561–564.
4 Chevaleyre V, Takahashi KA, Castillo PE. Endocannabinoid- mediated synaptic plasticity in the CNS. Annu Rev Neurosci 2006; 29: 37–76.5 Galve-Poperh I, Aguado T, Palazuelos J, Guzman M. The endocannabinoid system and neurogenesis in health and disease. Neuroscientist 2007; 13: 109–114.
6 Kreitzer AC, Regehr WG. Retrograde inhibition of presynaptic calcium influx by endogenous cannabinoids at excit- atory synapses onto Purkinje cells. Neuron 2001; 29: 717– 727.
7 Ohno-Shosaku T, Maejima T, Kano M. Endogenous cannabi- noids mediate retrograde signals from depolarized postsyn- aptic neurons to presynaptic terminals. Neuron 2001; 29: 729–738.
8 Wilson RI, Nicoll RA. Endogenous cannabinoids mediate retrograde signalling at hippocampal synapses. Nature 2001; 410: 588–592.
9 Yazulla S. Endocannabinoids in the retina: from marijuana to neuroprotection. Prog Retin Eye Res 2008; 27: 501–526.
10 Zabouri N, Bouchard JF, Casanova C. Cannabinoid receptor type 1 expression during postnatal development of the rat retina. J Comp Neurol 2011; 519: 1258–1280.
11 Lalonde MR, Jollimore CA, Stevens K, Barnes S, Kelly ME. Cannabinoid receptor-mediated inhibition of calcium signal- ing in rat retinal ganglion cells. Mol Vis 2006; 12: 1160– 1166.
12 Porcella A, Maxia C, Gessa GL, Pani L. The human eye ex- presses high levels of CB1 cannabinoid receptor mRNA and protein. Eur J Neurosci 2000; 12: 1123–1127.
13 Straiker AJ, Maguire G, Mackie K, Lindsey J. Localization of cannabinoid CB1 receptors in the human anterior eye and retina. Invest Ophthalmol Vis Sci 1999; 40: 2442–2448.
14 Yazulla S, Studholme KM, McIntosh HH, Deutsch DC. Im- munocytochemical localization of cannabinoid CB1 receptor and fatty acid amide hydrolase in rat retina. J Comp Neurol 1999; 415: 80–90.
15 Yazulla S, Studholme KM, McIntosh HH, Fan SF. Cannabi- noid receptors on goldfish retinal bipolar cells: electron- microscope immunocytochemistry and whole-cell record- ings. Vis Neurosci 2000; 17: 391–401.
16 Fan SF, Yazulla S. Biphasic modulation of voltage-depen- dent currents of retinal cones by cannabinoid CB1 receptor agonist WIN 55212–2. Vis Neurosci 2003; 20: 177–188.
3 Matsuda LA, Lolait SJ, Brownstein MJ, Young AC, Bonner TI. Structure of a cannabinoid receptor and functional expression of the cloned cDNA. Nature 1990; 346: 561–564.
4 Chevaleyre V, Takahashi KA, Castillo PE. Endocannabinoid- mediated synaptic plasticity in the CNS. Annu Rev Neurosci 2006; 29: 37–76.5 Galve-Poperh I, Aguado T, Palazuelos J, Guzman M. The endocannabinoid system and neurogenesis in health and disease. Neuroscientist 2007; 13: 109–114.
6 Kreitzer AC, Regehr WG. Retrograde inhibition of presynaptic calcium influx by endogenous cannabinoids at excit- atory synapses onto Purkinje cells. Neuron 2001; 29: 717– 727.
7 Ohno-Shosaku T, Maejima T, Kano M. Endogenous cannabi- noids mediate retrograde signals from depolarized postsyn- aptic neurons to presynaptic terminals. Neuron 2001; 29: 729–738.
8 Wilson RI, Nicoll RA. Endogenous cannabinoids mediate retrograde signalling at hippocampal synapses. Nature 2001; 410: 588–592.
9 Yazulla S. Endocannabinoids in the retina: from marijuana to neuroprotection. Prog Retin Eye Res 2008; 27: 501–526.
10 Zabouri N, Bouchard JF, Casanova C. Cannabinoid receptor type 1 expression during postnatal development of the rat retina. J Comp Neurol 2011; 519: 1258–1280.
11 Lalonde MR, Jollimore CA, Stevens K, Barnes S, Kelly ME. Cannabinoid receptor-mediated inhibition of calcium signal- ing in rat retinal ganglion cells. Mol Vis 2006; 12: 1160– 1166.
12 Porcella A, Maxia C, Gessa GL, Pani L. The human eye ex- presses high levels of CB1 cannabinoid receptor mRNA and protein. Eur J Neurosci 2000; 12: 1123–1127.
13 Straiker AJ, Maguire G, Mackie K, Lindsey J. Localization of cannabinoid CB1 receptors in the human anterior eye and retina. Invest Ophthalmol Vis Sci 1999; 40: 2442–2448.
14 Yazulla S, Studholme KM, McIntosh HH, Deutsch DC. Im- munocytochemical localization of cannabinoid CB1 receptor and fatty acid amide hydrolase in rat retina. J Comp Neurol 1999; 415: 80–90.
15 Yazulla S, Studholme KM, McIntosh HH, Fan SF. Cannabi- noid receptors on goldfish retinal bipolar cells: electron- microscope immunocytochemistry and whole-cell record- ings. Vis Neurosci 2000; 17: 391–401.
16 Fan SF, Yazulla S. Biphasic modulation of voltage-depen- dent currents of retinal cones by cannabinoid CB1 receptor agonist WIN 55212–2. Vis Neurosci 2003; 20: 177–188.
17 Straiker A, Sullivan JM. Cannabinoid receptor activation dif- ferentially modulates ion channels in photoreceptors of the tiger salamander. J Neurophysiol 2003; 89: 2647–2654.
18 Fan SF, Yazulla S. Retrograde endocannabinoid inhibition of goldfish retinal cones is mediated by 2-arachidonoyl glycerol. Vis Neurosci 2007; 24: 257–267.
19 Marc RE, Liu W. Fundamental GABAergic amacrine cell
circuitries in the retina: nested feedback, concatenated inhi- bition, and axosomatic synapses. J Comp Neurol 2000; 425: 560–582.
20 Protti DA, Gerschenfeld HM, Llano I. GABAergic and glycinergic IPSCs in ganglion cells of rat retinal slices. J Neurosci 1997; 17: 6075–6085.
21 Wässle H. Parallel processing in the mammalian retina. Nat Rev Neurosci 2004; 5: 747–757.
22 Wässle H, Boycott BB. Functional architecture of the mam- malian retina. Physiol Rev 1991; 71: 447–480.
23 Yang XF, Miao Y, Ping Y, Wu HJ, Yang XL, Wang Z. Mela- tonin inhibits tetraethylammonium-sensitive potassium channels of rod ON type bipolar cells via MT2 receptors in rat retina. Neuroscience 2011; 173: 19–29.
24 Zhao WJ, Zhang M, Miao Y, Yang XL, Wang Z. Melatonin potentiates glycine currents through a PLC/PKC signaling pathway in rat retinal ganglion cells. J Physiol (Lond) 2010; 588: 2605–2619.
25 Fohlmeister JF, Cohen ED, Newman EA. Mechanisms and distribution of ion channels in retinal ganglion cells: using temperature as an independent variable. J Neurophysiol 2010; 103: 1357–1374.
26 Naundorf B, Wolf F, Volgushev M. Unique features of action potential initiation in cortical neurons. Nature 2006; 440: 1060–1063.
27 Margolis DJ, Detwiler PB. Different mechanisms generate maintained activity in ON and OFF retinal ganglion cells. J Neurosci 2007; 27: 5994–6005.
28 Middleton TP, Protti DA. Cannabinoids modulate spontane- ous synaptic activity in retinal ganglion cells. Vis Neurosci 2011; 28: 393–402.
29 Fu H, Xiao JM, Cao XH, Ming ZY, Liu LJ. Effects of WIN55,212-2 on voltage-gated sodium channels in trigemi- nal ganglion neurons of rats. Neurol Res 2008; 30: 85–91.
30 Kim HI, Kim TH, Shin YK, Lee CS, Park M, Song JH. Anandamide suppression of Na+ currents in rat dorsal root ganglion neurons. Brain Res 2005; 1062: 39–47.
31 Jensen RJ, DeVoe RD. Comparisons of directionally selec- tive with other ganglion cells of the turtle retina: intracellular recording and staining. J Comp Neurol 1983; 217: 271–287.
32 Karschin A, Lipton SA. Calcium channels in solitary retinal ganglion cells from post-natal rat. J Physiol (Lond) 1989;
circuitries in the retina: nested feedback, concatenated inhi- bition, and axosomatic synapses. J Comp Neurol 2000; 425: 560–582.
20 Protti DA, Gerschenfeld HM, Llano I. GABAergic and glycinergic IPSCs in ganglion cells of rat retinal slices. J Neurosci 1997; 17: 6075–6085.
21 Wässle H. Parallel processing in the mammalian retina. Nat Rev Neurosci 2004; 5: 747–757.
22 Wässle H, Boycott BB. Functional architecture of the mam- malian retina. Physiol Rev 1991; 71: 447–480.
23 Yang XF, Miao Y, Ping Y, Wu HJ, Yang XL, Wang Z. Mela- tonin inhibits tetraethylammonium-sensitive potassium channels of rod ON type bipolar cells via MT2 receptors in rat retina. Neuroscience 2011; 173: 19–29.
24 Zhao WJ, Zhang M, Miao Y, Yang XL, Wang Z. Melatonin potentiates glycine currents through a PLC/PKC signaling pathway in rat retinal ganglion cells. J Physiol (Lond) 2010; 588: 2605–2619.
25 Fohlmeister JF, Cohen ED, Newman EA. Mechanisms and distribution of ion channels in retinal ganglion cells: using temperature as an independent variable. J Neurophysiol 2010; 103: 1357–1374.
26 Naundorf B, Wolf F, Volgushev M. Unique features of action potential initiation in cortical neurons. Nature 2006; 440: 1060–1063.
27 Margolis DJ, Detwiler PB. Different mechanisms generate maintained activity in ON and OFF retinal ganglion cells. J Neurosci 2007; 27: 5994–6005.
28 Middleton TP, Protti DA. Cannabinoids modulate spontane- ous synaptic activity in retinal ganglion cells. Vis Neurosci 2011; 28: 393–402.
29 Fu H, Xiao JM, Cao XH, Ming ZY, Liu LJ. Effects of WIN55,212-2 on voltage-gated sodium channels in trigemi- nal ganglion neurons of rats. Neurol Res 2008; 30: 85–91.
30 Kim HI, Kim TH, Shin YK, Lee CS, Park M, Song JH. Anandamide suppression of Na+ currents in rat dorsal root ganglion neurons. Brain Res 2005; 1062: 39–47.
31 Jensen RJ, DeVoe RD. Comparisons of directionally selec- tive with other ganglion cells of the turtle retina: intracellular recording and staining. J Comp Neurol 1983; 217: 271–287.
32 Karschin A, Lipton SA. Calcium channels in solitary retinal ganglion cells from post-natal rat. J Physiol (Lond) 1989;
418: 379–396.
33 Clark BD, Kurth-Nelson ZL, Newman EA. Adenosine-
evoked hyperpolarization of retinal ganglion cells is mediat- ed by G-protein-coupled inwardly rectifying K+ and small conductance Ca2+-activated K+ channel activation. J Neurosci 2009; 29: 11237–11245.
34 Ettaiche M, Heurteaux C, Blondeau N, Borsotto M, Tinel N, Lazdunski M. ATP-sensitive potassium channels KATP in reti- na: a key role for delayed ischemic tolerance. Brain Res
evoked hyperpolarization of retinal ganglion cells is mediat- ed by G-protein-coupled inwardly rectifying K+ and small conductance Ca2+-activated K+ channel activation. J Neurosci 2009; 29: 11237–11245.
34 Ettaiche M, Heurteaux C, Blondeau N, Borsotto M, Tinel N, Lazdunski M. ATP-sensitive potassium channels KATP in reti- na: a key role for delayed ischemic tolerance. Brain Res
2001; 890: 118–129.
35 Koeberle PD, Wang Y, Schlichter LC. Kv1.1 and Kv1.3
channels contribute to the degeneration of retinal ganglion cells after optic nerve transection in vivo. Cell Death Differ 2010; 17: 134–144.
36 Lipton SA, Tauck DL. Voltage-dependent conductances of solitary ganglion cells dissociated from the rat retina. J Phys- iol (Lond) 1987; 385: 361–391.
35 Koeberle PD, Wang Y, Schlichter LC. Kv1.1 and Kv1.3
channels contribute to the degeneration of retinal ganglion cells after optic nerve transection in vivo. Cell Death Differ 2010; 17: 134–144.
36 Lipton SA, Tauck DL. Voltage-dependent conductances of solitary ganglion cells dissociated from the rat retina. J Phys- iol (Lond) 1987; 385: 361–391.
